As I went back there to see whether I could pick up some ideas, I realized that the post in question is one of the best I have ever written. This discourages me from attempting to out-do it here: I will thus direct there those of you who want to learn about the underlying physics or are curious about the latest direct searches for a fourth-generation quark. Here, instead, I will stick with a discussion of the interesting preprint, which is titled "Four Statements About the Fourth Generation". The paper is a summary of a workshop held at CERN last September. Its authors are B. Holdom, W. Hou, T. Hurth, M. Mangano, S. Sultansoy, and G. Ünel.
The four facts discussed in the paper are distilled wisdom coming from years of studies and investigations, and summarizing a summary is a despicable occupation. I will justify it by trying to give nothing for granted and explaining things in a way my aunts could understand (sorry, I appear to be out of grandmothers, but I assure you my aunts are a good replacement as far as physics understanding is concerned). So let me first of all refresh your memory with some basic facts about quarks and leptons.
Introduction: learning to count to three
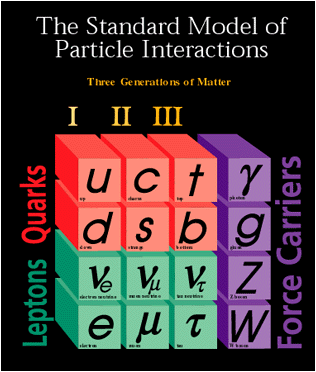
I said a trio of quarks because each of the quarks in the picture -u,d,s,c,b,t- must be actually understood as three different quarks, each carrying a different "strong charge", one we call colour; the corresponding theory describing the interaction of colour charge is called Quantum Chromo-Dynamics, or QCD for insiders. Because of colour, you can have green, red, and blue quarks. They organize into observable particles by binding into colourless objects: three together -one of each color- make a baryon, while a quark-antiquark pair makes a meson. Baryons and mesons are the two known ways by means of which nature hides colour from us -colour is an internal charge that we never directly experience.

The scheme discussed above is neat: colour charge keeps quarks bound together within protons and neutrons, and also holds together scores of the latter within tightly-packed nuclei. It is the strong force what creates structure in the Universe! However, it seems we just need up- and down-type quarks after all. What is the use of the other four, then ?
Who ordered that ?
The above question is ill-posed because of historical reasons: in fact, we did not discover up- and down-quarks first, but rather we got to know for a fact that protons and neutrons are composed of quarks only after we discovered the fourth quark, so-called charm, in what was later famously dubbed "November Revolution", in 1974. Until then, quarks had not been considered real entities, but just a mathematical construct capable of cataloging all known hadrons into simple structures, called multiplets. The first block of an extra generation of leptons, as a matter of fact, had been found much earlier.

Two generations remained such only for three years after the November Revolution, because in 1977 the bottom quark discovery at the Tevatron made it clear that there was a third one to deal with. In truth, a third-generation lepton had been discovered two years earlier, by Martin Perl at Stanford; but Perl's discovery remained controversial way past the bottom quark discovery. Regardless of the nature of the tau-lepton, by 1977, physicists had to acknowledge that the number of quark and lepton generations were at least three.
Since then, physicists have grown to love the setup. Three is a magic number for several reasons; for instance, I have just explained above how three colour charges are needed to describe Quantum Chromo-Dynamics, the theory that explains the binding of quarks inside protons, neutrons, and all other hadrons we can produce and study in accelerator collisions; and three is also the ratio between the electric charge of electrons and that of down-type quarks (I could sell this to you as an independent fact, but in reality it is deeply intertwined with the former one).
In any case, three generations of quarks and leptons appear a very clean arrangement, once one is forced to acknowledge that one generation is not the way Nature (the bitch, not the magazine) has chosen for us. With the CDF discovery of the top quark in 1994, the picture was now complete. Or was it ?
Why not more than three ?
There are no fundamental reasons why there cannot be a fourth generation of quarks and leptons, so it makes sense to put together a few agreed-upon facts on the matter. That is what the paper by Holdom et al. does.
The first statement made in the paper is that there is no real evidence against the existence of a fourth generation of matter. The measurement of electroweak physics observables performed at the LEP collider at CERN in the nineties, and a few additional precise results from the Tevatron, can be used to stringently test the Standard Model, and among other things they also constrain the unknown mass of the Higgs boson; but they cannot easily conspire to demonstrate that there are only three families of quarks and leptons.

The CKM matrix is nothing but a parametrization of the probability that a quark turns into ("mixes" with) one of a different kind, a piece of magic allowed by weak interactions. If you have six quarks in three sets of two, and you only allow quarks of the up-type to mix with ones of the down-type as the Standard Model dictates, you can write these "mixing parameters" in a three-by-three matrix, as shown on the left below. The V symbols denote different elements of the matrix, the mixing parameters.
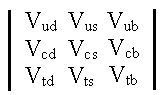
After considering the above issues and others, the authors of the paper conclude that a fourth generation can exist in Nature without contradicting existing measurements. That is valuable information, in particular if you consider that our bible rather argued against this, as I noted above.
The second statement is even more intriguing than the first: the authors say that a fourth generation of fermions would not just be possible, but it would also be useful to address and provide an explaination to some of the theoretical questions that the present model brings along.
One issue that is briefly considered is the fact that a fourth generation of quarks and leptons could allow for the right size of a quantum-mechanical effect called CP violation, a phenomenon which could have originated a Universe containing more matter than antimatter, as the one we seem to be living in. This is an exciting possibility, since so far physicists have been struggling with a solution of that question: the Standard Model does not explain how such an asymmetry to have originated, since the amount of CP violation it allows for is tiny.
Another interesting point is the possible onset of Flavor Democracy. The three families of quarks and leptons have widely varied masses, and this means they couple to the Higgs boson with greatly different strenghts; this is a puzzling feature of the Standard Model. In a Flavor-Democratic world, instead, all of them would have the same coupling, and the different in masses would be due to perturbations; such a thing is not feasible with three generations because of the observed difference of mass of third-generation quarks and leptons, but with a fourth generation the scheme could work.
A point about dark matter is also made in the paper. With a fourth generation one could imagine new particles that would conveniently fill in the matter gap of the matter-energy budget of the Universe; some examples are made in the paper. I think any new physics model nowadays must somehow allow for a dark matter candidate, so this latter piece of information fails to impress me much; however, it is good to know that the one we are discussing passes the test.
The third statement made in the paper is that a fourth generation of quarks and leptons can provide a way to "explain away" some of the emerging effects seen in recent experimental data. One example is the "excess" of events seen by CDF in its search for a fourth-generation quark in its Run II dataset: the data at high values of reconstructed mass in the graph below.

In the plot you can see the reconstructed mass of CDF fourth-generation quark decay candidates (black points with error bars) compared with the sum of predicted backgrounds, and a possible fourth-generation quark signal (with 280 GeV mass) in yellow. While the "bump" above background sitting at about 400 GeV is really insignificant from a statistical standpoint, the authors do mention it in their paper. I was rather surprised by this slip into wild speculation mode, especially since I know personally one of the authors (Michelangelo Mangano) as one very rigorous theorist. But I have to admit that in a section about emerging hints of a SM breakdown one is expected to let go one way or another with similar speculative remarks.
And since I love to speculate, here is the display of one of the events belonging to the bump shown above: it is a very spectacular, energetic collision, yielding a 150 GeV muon (in green), a large energy inbalance (labeled by a red arrow, most likely due to an escaping neutrino), plus several hadronic jets. If this was the decay of a heavy quark, its mass would be of 450 GeV: more than twice the mass of the heaviest known quark, the top. The drawing shows what you would observe if you sat along the proton-antiproton beam line and looked at the CDF detector face on. The inner segments represent charged track trajectories, while the outer colored boxes describe the energy deposited by particles in devices called calorimeters.

But let me get back to the preprint I have been summarizing. The fourth, and final statement made in the paper is that the LHC has the potential to find, or fully exclude, a fourth generation of quarks and leptons. This is not hard to believe, since QCD is democratic with quark flavor, so even fourth-generation ones would be produced aplenty in LHC collisions. CMS and ATLAS could easily exclude them up to 1 TeV, which is about the maximum mass that fourth-generation quarks could have to avoid breaking partial wave unitarity, which is another kind of "probability conservation", similar to the one I discussed earlier.
A point about Higgs boson prospects is made in the paper at this point. As I explained in some detail elsewhere, a fourth-generation quark -especially a heavy one- would contribute to the production of Higgs bosons; but it would also contribute to the possible decays, with an altogether rather unclear picture emerging. For light Higgs boson masses, one could get reduced rates of gamma-gamma final states, increases of the two-gluon decay, decays to invisible leptons enhanced. In summary, it is basically anybody's guess whether a fourth generation of fermions would help us or hinder us in our Higgs searches.
In conclusions, the study seems to make the case for the possibility that three generations of matter are not the end of the story. I find four not particularly attractive as an alternative to three. What is your guess ? You can in principle pick any number from three to 16. Not more than that, though, since then another fundamentum of particle physics would break down: with more than 16 generations of quarks, the properties of QCD would become completely different from what we observe.
Yes, I know what you are thinking: if we have to renounce to the elegant economicity of a single generation, and once three are also too few, an appealing alternative would be an infinite number of replicas. Well, unfortunately that is not a possibility. But do not despair: if we do not manage to appreciate beauty in the Standard Model, maybe it is because we still know too little about it.
Comments