We can also "see" showers of secondary particles from cosmic rays thanks to the Cherenkov light they produce. Cherenkov light is emitted when charged particles travel in a medium at speeds higher than the speed of light itself! Light, in fact, slows down a little when it traverses a medium; energetic particles do not, so they create a conic "shock wave" similar to the boom of supersonic airplanes.
By imaging these light cones, ground-based instruments watching the sky detect showers originated from charged particles, as well as ones produced by very energetic gamma rays. One such instrument is the MAGIC detector, made by two 17-meter telescopes operating in synchrony. It is located on top of the island of La Palma, in the Canary islands.

(Above: The MAGIC telescopes)
The information we obtain by studying cosmic rays originated by protons and light nuclei is limited by the fact that those particles are electrically charged, so they got deflected by the galactic magnetic fields they traveled through: their direction of arrival does not allow us to pinpoint their true origin. Gamma rays and neutrons, on the other hand, are electrically neutral, so they travel along straight paths, betraying their emitting source if observed. But there's a big difference between them: while gamma rays may reach us by traveling for billions of years after crossing our entire Universe, neutrons are unstable particles when they are stripped away from atomic nuclei and left alone: they on average decay in 15 minutes in their own reference system!
Einstein's theory of special relativity comes to the rescue: if a neutron shot toward us at high energy by a source within our galaxy travels at a speed very close to the speed of light in our reference frame, its own time runs way more slowly than ours. Because of that "time dilation" effect, very energetic neutrons make it to Earth before decaying, and carry directional information from as far as the other side of our own galaxy.
Astrophysicists today rely on two additional kinds of cosmic messengers. The first are neutrinos. Neutrinos are neutral, lightweight elementary particles produced by the fusion processes that take place in the interior of stars. Every second our Sun shines with two hundred billion billion billion billion neutrinos. That’s a lot of neutrinos: a trillion of those pass through your eyeballs alone! Not to worry: they interact extremely feebly with matter, so your eyeballs are safe - but that also makes their detection quite challenging. We may "see" them by deploying a very large volume of water or some other transparent medium in underground tanks that are watched by light-sensitive detectors. When a neutrino hits a nucleus of the medium, it produces particles that release Cherenkov light as they whiz through.

(Above: circles of Cherenkov light are detected by the photomultipliers instrumenting the Super-Kamiokande detector tank following a neutrino interaction)
The largest detector for cosmic neutrinos is currently the ICECUBE experiment in Antarctica. ICECUBE consists of a widely spaced lattice of 86 holes drilled from the surface into transparent underground ice down to a depth of 2450 meters; a long bead-like string of 60 light detectors is inserted in each hole. The system collectively watches a volume of over a cubic kilometer of ice. Neutrinos tell us a whole lot about the complex phenomena that take place in the core of stars.

(Above: layout of the ICECUBE detector)
A curious fact about the surprising neutrinos is that, due to their extremely feeble interaction, they are not slowed down in traversing matter as light does: so they out-run everything else! Indeed, when 168,000 years ago an exploding supernova in the Small Magellanic Cloud produced an enormous number of neutrinos in addition to a huge blast of light and matter, this set up a race between neutrinos and photons. In 1987, after covering a distance of 168 thousand light years, 25 neutrinos were eventually detected by neutrino detectors on Earth, some four hours before the light of the explosion could also reach us!
By detecting neutrinos in association with cosmic rays, radio waves, visible light, and X and gamma rays, we have acquired the means to do what is today called multi-messenger astrophysics: the information we collect about energetic emissions of radiation from distant sources has thus multiplied. And that's not all! The most recent cosmic messengers we rely upon today, in our continuing quest to understand our Universe, are a true game-changer: their name is gravitational waves.
While gravity feels like a strong force to us, due to the large amount of matter pulling us toward the center of our planet, it is extremely weak if compared with the electromagnetic force. Take two protons: they are the building blocks of matter, so they make a good reference unit. Protons are charged and massive; they attract each other because of their mass, and repel because of their equal electric charge. The repulsive electromagnetic force they feel, however, is about a billion billion billion billion times stronger than their gravitational attraction! Because of that, while the motion of two electrically charged bodies past one another generates an easily detectable electromagnetic wave, waves produced by a gravitational interaction between two massive bodies are completely undetectable - unless the mass times inverse-square distance of those bodies is humongous.
Who said humongous mass and inverse distance? We are clearly speaking of close-orbiting black holes or neutron stars, which are objects we would love to learn more about. Black holes are not uncommon: most galaxies contain a super-massive black hole in their center: the 2020 Nobel prize in Physics indeed went to Reinhard Genzel and Andrea Ghez for their discovery of the giant black hole at the center of our own galaxy, the Milky Way. Smaller ones move around, gobbling up stars and planets that cross their path. And when two black holes meet a spiraling dance sets up, which concludes in their merging into a single one. These catastrophically energetic events generate ripples in space-time, deformations that propagate at the speed of light in all directions.
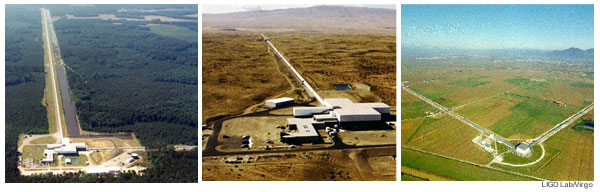
(Above: the Ligo, Ligo-Hanford, and Virgo gravitational antennas)
The LIGO and VIRGO gravitational antennas, located respectively in the US and Italy, exploit the self-interference of a laser beam that travels back and forth along two orthogonal arms of a multi-kilometer light path, reflecting onto carefully amortized, suspended mirrors. When a gravitational wave crosses our planet, it stretches one of the two arms of the instrument by a tiny amount - a displacement much smaller than the diameter of a proton! - and shrinks the other by a similar distance. This is enough to produce a tell-tale signal in the time evolution of the interference pattern of the light beam, allowing detection of the wave train; and if seen by more than one antenna, the wave's arrival direction in the sky can also be pinpointed.
Because of their reliance on complementary physics phenomena to light and particle detectors, gravitational wave antennas have opened an entirely new way of exploring the Universe. Those instruments promise to greatly expand our knowledge about location, abundance, and characteristics of black holes, as well as to probe the physics of neutron stars. Indeed, very recently the first combined observation of gravitational waves and gamma rays, emitted together when two massive neutron stars collided and merged, have been reported.
Today experiments and observatories all over the world, underground detectors, and instrumented satellites work together in a global network, providing a real-time survey of the cosmos, and amassing data on the Universe, its structure, and its evolution. This is a truly exciting time to be an astrophysicist!
---
Tommaso Dorigo (see his personal web page here) is an experimental particle physicist who works for the INFN and the University of Padova, and collaborates with the CMS experiment at the CERN LHC. He coordinates the MODE Collaboration, a group of physicists and computer scientists from eight institutions in Europe and the US who aim to enable end-to-end optimization of detector design with differentiable programming. Dorigo is an editor of the journals Reviews in Physics and Physics Open. In 2016 Dorigo published the book "Anomaly! Collider Physics and the Quest for New Phenomena at Fermilab", an insider view of the sociology of big particle physics experiments. You can get a copy of the book on Amazon, or contact him to get a free pdf copy if you have limited financial means.

Comments